.
Before LIGO’s big day, Nature chronicles the search for Einstein's predicted ripples in space-time.
-
Scientists at the Laser Interferometer Gravitational-Wave Observatory (LIGO) are expected to announce on 11 February that they have detected gravitational waves: ripples in space-time that Albert Einstein predicted a century ago. LIGO, which is US-led, has sites in Hanford, Washington and Livingston, Louisiana. Nature takes a pictorial look at the quest to track down of one of the most elusive quarries in physics.
.
June 1916: Einstein predicts ripples in the firmament
.
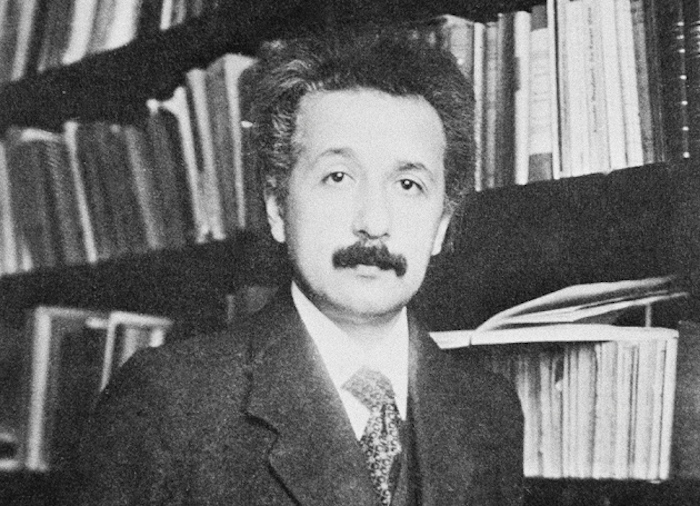
After formulating his general theory of relativity, which holds that gravity is a distortion in space and time around massive objects, Albert Einstein (pictured) pondered what would happen when a mass is shaken. His answer, published a year after the theory, was that space-time would ripple and produce ‘gravitational waves’ moving outward from the mass at the speed of light1. He and other physicists immediately began arguing about whether the predicted waves were real, or just artefacts of the new mathematics involved. Einstein himself changed his mind several times.
-
June 1969: a detection is claimed
.
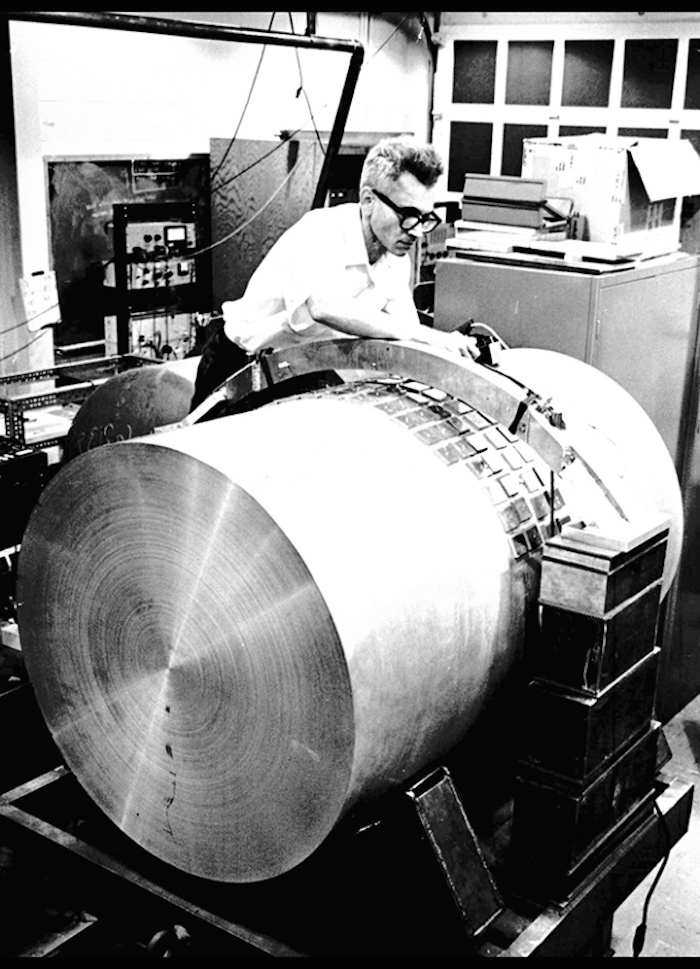
Joseph Weber (pictured), a physicist at the University of Maryland in College Park, believed that gravitational waves were real. In 1969, he announced that he had found them with a detector of his own invention: an aluminium cylinder, about 2 metres long and 1 metre in diameter, that ‘rang’ when it was struck by such a wave2. His result was never replicated, and was eventually rejected by nearly everyone except Weber himself. Nonetheless, his work drew many other researchers into the gravitational wave field.
-
1974: neutron stars provide a step forward
.
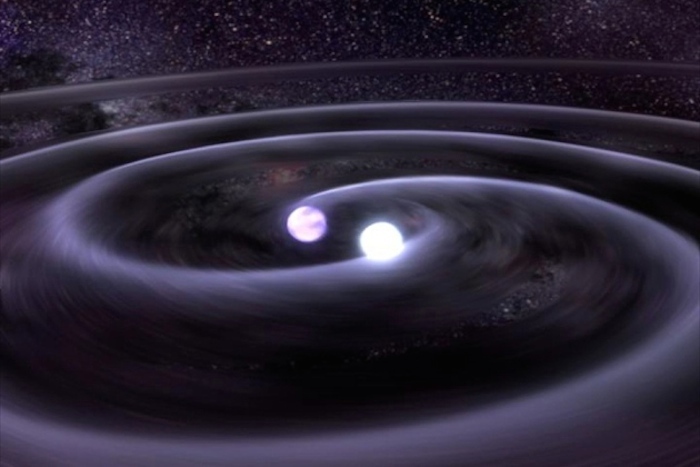
A more widely believed finding came from Joseph Taylor and his graduate student Russell Hulse. Working at the University of Massachusetts Amherst, they discovered3 the first known binary pulsar, dubbed PSR B1913+16 (artist's impression, pictured), in 1974. It consisted of two neutron stars whipping around one another in a close orbit, and also spiralling inwards at exactly the rate predicted by Einstein’s theory — if gravitational waves were carrying away their energy. Viewed as an indirect observation of gravitational waves, the find won Hulse and Taylor the 1993 Nobel Prize in Physics, with the citation: “for the discovery of a new type of pulsar, a discovery that has opened up new possibilities for the study of gravitation”.
-
1990: enter LIGO
.
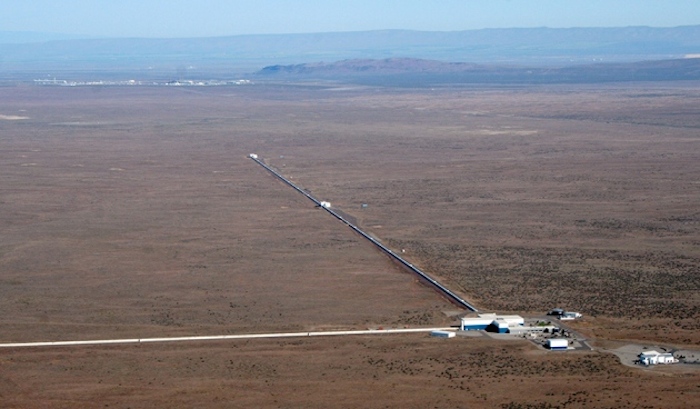
When scientists at the Massachusetts Institute of Technology and the California Institute of Technology submitted plans for huge detectors to find gravitational waves using a technique known as laser interferometry, many researchers were strongly opposed to the project. They feared that it would divert huge amounts of money from other research and — moreover — never find anything. But the US National Science Foundation (NSF) approved the construction of LIGO in 1990, and in 1992 selected sites for the experiment’s twin detectors: Hanford, Washington (pictured), and Livingston, Louisiana. The facilities were completed in 1999 and started collecting data in 2001. In 2010, the detectors were shut down for upgrades, having found nothing.
-
March 2014: primordial wave claim
.
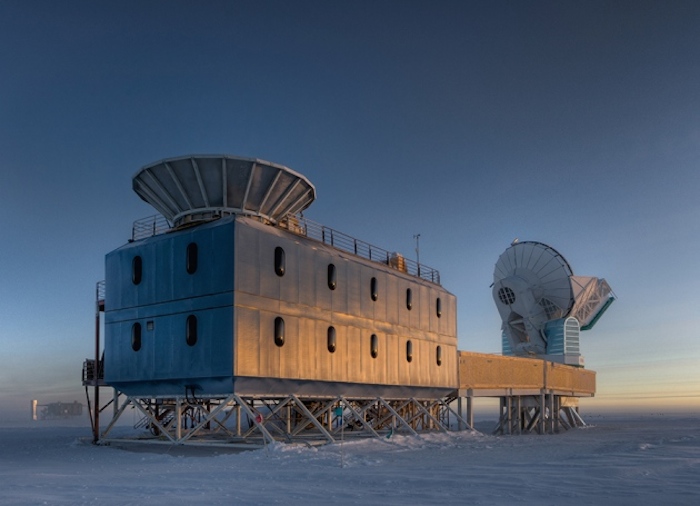
In 2014, a different type of evidence for gravitational waves was claimed, by the collaboration behind a South Pole-based instrument called BICEP2 (facility, pictured). The BICEP2 researchers said that they had found ‘B modes’ — subtle microwave signals that they believed to be produced by ‘primordial’ gravitational waves generated by the Big Bang. But their analysis was subsequently revealed to be a mistake: the signal was the result of interstellar dust particles in our own Galaxy.
-
September 2015: Advanced LIGO
.
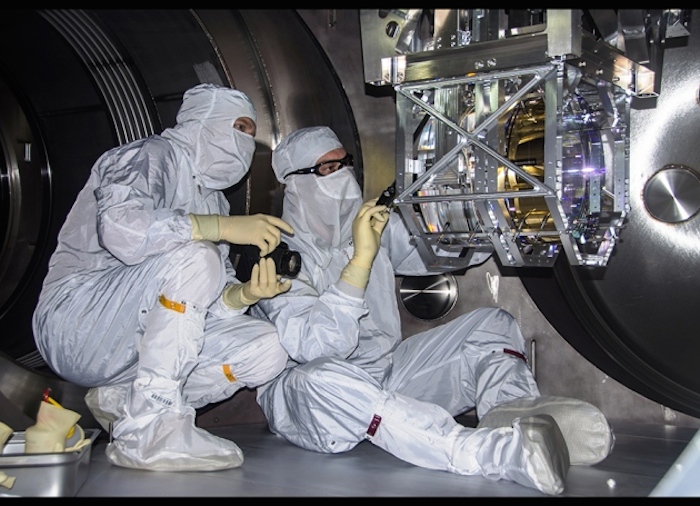
A hugely upgraded LIGO began its first observing run last September, with the ability to search for gravitational waves in a much greater volume of space than its predecessor could. Advanced LIGO finished its first period of operation in January 2016. Researchers around the world expect to find out on 11 February whether the long-sought gravitational waves have finally been observed. (Pictured: an inspection at the Livingston detector.)
Quelle: nature
---
Erstmals Nachweis von Gravitationswellen gelungen Es ist ein wissenschaftlicher Paukenschlag: US-Forscher haben die vor 100 Jahren von Albert Einstein vorhergesagten Gravitationswellen erstmals nachgewiesen. Damit ist ihnen der Nobelpreis sicher.
Wenn Wissenschaftler zeitgleich zu einer Presskonferenz in Washington, Moskau, Pisa und Hannover einladen, dann haben sie Großes zu verkünden. Jetzt ist es offiziell: Es ist erstmals gelungen, eine Gravitationswelle direkt nachzuweisen.
Es war ein kleines Zittern des Raumes, aber ein großes Beben für die Physik, als am 14. September letzten Jahres eine Gravitationswelle über die Erde hinwegrauschte. Kein Mensch bemerkte etwas davon, nur zwei Messinstrumente namens Advanced Ligo (Laser-Interferometer Gravitationswellen-Observatorium) in den USA registrierten eine kurzzeitige Verbiegung des Raumes.
Das Signal, ausgelöst von zwei miteinander verschmelzenden Schwarzen Löchern, dauerte nur etwa eine Zehntelsekunde, und doch erschüttert es die Welt der Physiker. Genau hundert Jahre nach Einsteins Vorhersage wurde hiermit die Existenz von Gravitationswellen erstmals zweifelsfrei bewiesen.
Diese nobelpreiswürdige Entdeckung galt als letzter fehlender Baustein der Allgemeinen Relativitätstheorie, und sie öffnet ein völlig neues Beobachtungsfenster ins Universum. Möglich wurde diese Entdeckung durch Entwicklungen von Forschern der Universität Hannover und dem Max-Planck-Institut für Gravitationsphysik in Golm in Kooperation mit der Universität Glasgow.
Im Internet häuften sich die Gerüchte
Die Gerüchteküche brodelte bereits seit September, als der amerikanische Physiker Lawrence Krauss, der selbst nicht zur Advanced Ligo-Collaboration gehört, via Twitter die Sensation andeutete. Doch schnell wurde dementiert. Heute ist klar, warum. Die Gravitationsforscher wollten nicht in dieselbe Falle tappen wie ein Jahr zuvor ihre Kollegen des Projekts Bicep-2.
Die hatten mit großem Medienrummel behauptet, in der kosmischen Hintergrundstrahlung den Fingerabdruck von Gravitationswellen aus dem Urknall gemessen zu haben. Eine nachträgliche Datenanalyse zeigte dann jedoch, dass die Himmelsforscher einem Messfehler aufgesessen waren. Bei Advanced Ligo haben die Forscher keine Zweifel.
"Das Signal war so stark, dass überhaupt keine aufwendige statistische Analyse nötig war, erklärt Karsten Danzmann, Leiter des Gravitationswellendetektors Geo600 in Ruthe bei Hannover. Vor allem aber tauchte das Signal in zwei identischen Advanced-Ligo-Anlagen auf, die 3000 Kilometer voneinander entfernt in Livingston (Louisiana) und Hanford (Washington) arbeiten. Ein technischer Fehler ist damit so gut wie ausgeschlossen.
Überdies wurde im Internet kolportiert, dass ein Mitglied der Advanced Ligo-Collaboration gezielt und ohne Wissen der anderen Mitarbeiter eine Störung in die Anlagen einbringen kann, die ein echtes Signal aus dem Kosmos vortäuscht.
So sollte die Zuverlässigkeit der Datenauswertung auf die Probe gestellt werden. Das war jedoch am 14. September nicht der Fall, denn "die Anlagen befanden sich in der Phase der Inbetriebnahme, während die gezielten Blindsignale nur im Routinebetrieb eingespeist werden", erklärt Danzmann.
Die Wissenschaftler sind elektrisiert
Die Advanced-Ligo-Forscher waren aber von der Deutlichkeit des Messsignals so überrascht, dass sie es erst gar nicht als echt ansehen konnten. "Es ist fast zu schön, um wahr zu sein", schwärmt der Hannoveraner Forscher. Um zu verstehen, was die Wissenschaftler an ihrer Entdeckung so fasziniert, muss man ein Jahrhundert in die Vergangenheit zurückgehen.
Im November 1915 hatte Albert Einstein seine Allgemeine Relativitätstheorie vollendet. Nach ihr ist die Schwerkraft, physikalisch Gravitation genannt, eine Eigenschaft von Raum und Zeit. Jede Art von Materie krümmt den Raum um sich herum, und andere Körper sowie Lichtstrahlen müssen diesen Verbiegungen folgen.
Der Mond umkreist die Erde, weil die beiden Himmelskörper den umgebenden Raum eindellen wie schwere Kugeln ein gespanntes Gummituch und sich in diesen Mulden umeinander bewegen. Die Schwerkraft bestimmt das Geschehen im All in gleicher Weise wie auf der Erde. Deshalb gilt: Auch ein zu Boden fallendes Glas folgt der Raumkrümmung.
Mitte des Jahres 1916 kam Einstein zudem zu der Erkenntnis, dass es auch Wellen der Gravitation geben muss. Sie entstehen, wenn Himmelskörper sich beschleunigt bewegen. Dann versetzen sie den Raum in Schwingungen, die sich mit Lichtgeschwindigkeit ausbreiten. Man kann sie sich ähnlich vorstellen wie Wellen auf der Oberfläche eines Sees, in den man einen Stein geworfen hat.
Gravitationswellen kreuzen überall durchs All
Rauscht eine solche Gravitationswelle über die Erde hinweg, so staucht und dehnt sie kurzzeitig den Raum, das heißt, die Abstände zwischen allen Objekten ändern sich. Die Form einer Kugel beispielsweise würde wie ein mit Wasser gefüllter Ballon zwischen der eines Rugby-Balls und einer Kugel hin- und herschwingen.
Da das Universum voll ist mit Himmelskörpern, die sich beschleunigt bewegen, kreuzen immer und überall Gravitationswellen durchs All. Sie treffen auch auf die Erde.
Aber der Raum ist extrem steif, wie die Physiker gerne sagen. Gravitationswellen verbiegen eine Strecke vom Durchmesser der Erde gerade einmal um einen Bruchteil eines Atomdurchmessers. Einstein wusste das und hielt deshalb den Nachweis dieser Wellen für ausgeschlossen. Das Unterfangen erscheint wirklich unmöglich, dennoch ist es jetzt gelungen.
Hierfür mussten die empfindlichsten Instrumente gebaut werden, die es je gab, sogenannte Laser-Interferometer. Hierin wird ein Laserstrahl mit einem Spiegel in zwei Strahlen aufgespalten, in zwei gleichlange Röhren geleitet und nach dem Umlenken an Spiegeln wieder in einem Punkt zusammengeführt. Läuft eine Gravitationswelle über diese Anlage hinweg, so ändern sich kurzzeitig die Längen der beiden Strahlen, was sich in einem Flackern im Punkt ihrer Überlagerung äußert.
Anforderungen an die Technik sind extrem hoch
Von der ersten Idee für ein solches Interferometer im Jahre 1970 bis zur Realisierung vergingen rund dreißig Jahre. Zu Beginn dieses Jahrzehnts gingen drei Anlagen in Betrieb: der deutsch-britische Detektor Geo600 mit einer Länge von 600 Metern für die beiden Teilstrahlen sowie die beiden amerikanische Advanced-Ligo-Antennen mit einer Armlänge von jeweils vier Kilometern. Sie wurden 1992 von Kip Thorne und Ronald Drever (beide California Institute of Technology) sowie Rainer Weiss (Massachusetts Institute of Technology) gegründet.
Das Aufspüren der erwarteten winzigen Längenänderung erfordert extreme Anforderungen an die Stabilität des Laserstrahls und die erschütterungsfreie Lagerung der gesamten Anlage. Um sie zu gewährleisten, müssen die Forscher ständig die Grenzen des technisch Machbaren überschreiten. Hier haben die Hannoveraner Physiker Pionierarbeit geleistet und unter anderem das weltweit stabilste Lasersystem sowie eine neue Aufhängung für die Interferometerspiegel entwickelt.
Diese Elemente wurden in einer fünfjährigen Umbauphase in Advanced Ligo implantiert, wodurch die Reichweite um das Zehnfache und damit die Anzahl der beobachtbaren Galaxien um das Tausendfache zugenommen hat. Das brachte den Durchbruch.
Direkt an dem Tag, an dem die beiden Antennen im September letzten Jahres wieder in Betrieb gingen, rauschte – welch ein Glück – die Welle über die beiden Advanced Ligos hinweg. Geo600 lief gerade wegen eines technischen Problems nicht.
Darum sind Schwarze Löcher tatsächlich schwarz
Das nur rund eine Zehntel Sekunde lang andauernde Signal enthält wie ein Fingerabdruck ungeahnte Informationen. Es passt genau zu einem Typ von Ereignissen, die Theoretiker mit Supercomputer simuliert haben: die Vereinigung von zwei Schwarzen Löchern.
Ein Schwarzes Loch entsteht, wenn ein sehr massereicher Stern am Ende seines Lebens seinen Brennstoff verbraucht hat. Dann bricht sein Zentralbereich in sich zusammen, während die äußere Hülle abgesprengt wird und als Supernova aufleuchtet.
Der kollabierende Sternrest wird aber immer kompakter und verbiegt den umgebenden Raum immer mehr, bis die Krümmung theoretisch unendlich groß wird: Dieses Gebiet schnürt sich gewissermaßen vom Rest des Universums ab. Es ist jedoch halbdurchlässig: Von außen fällt alles hinein, aber es kommt nichts mehr heraus – auch Licht nicht. Deswegen sind diese Raumlöcher tatsächlich schwarz.
Da es im Universum sehr viele Doppelsterne gibt, muss es auch Doppel-Schwarze-Löcher geben, die sich gegenseitig umkreisen. Diese senden nach Einstein Gravitationswellen aus. Dadurch verlieren sie Energie, was sich darin äußert, dass sie sich auf einer spiralförmigen Bahn einander annähern und schließlich zusammenstoßen.
Zwei Schwarze Löcher verschmelzen miteinander
Nach der Relativitätstheorie verschmelzen hierbei zwei Raumbereiche mit unendlich starker Krümmung, in deren Zentren noch die Sternreste ruhen. "Unsere Computersimulationen sagen voraus, dass das neu entstehende Schwarze Loch kurzzeitig schwingt wie ein Ballon, bevor es zur Ruhe kommt", erklärt Alessandra Buonanno, Direktorin am MPI für Gravitationsphysik. Nur Gravitationswellen können über diese exotischsten und energiereichsten Vorgänge im Universum Auskunft geben, denn hierbei wird kein Lichtblitz oder Ähnliches frei.
"Genau dieses Szenario haben wir jetzt gesehen", sagt Danzmann, "es ist wie aus dem Lehrbuch." Nach derzeitiger Datenanalyse erzeugten das Signal vom 14. September zwei 1,3 Milliarden Lichtjahre entfernte Schwarze Löcher, die Materie mit jeweils 29 beziehungsweise 36 Sonnenmassen beinhalteten. Als sie verschmolzen, wurde Materie mit insgesamt drei Sonnenmassen komplett in Energie umgewandelt und in Form der Gravitationswellen abgegeben.
Damit strahlten diese beiden Schwergewichte in der kurzen Zeitspanne von einer Zehntelsekunde mehr Energie ab als alle Sterne im Universum zusammen. Auch hier steckt wieder Einstein mit seiner berühmten Formel E=mc2 drin. Sie besagt, dass Energie E und Materie m wesensverwandt sind und sich ineinander umwandeln können.
Mit den zwei Advanced-Ligo-Antennen lässt sich die Gravitationswellenquelle am Himmel nur sehr ungenau orten, dafür wären drei oder besser vier Antennen nötig. Die Forscher setzen deswegen ihre Hoffnung auf die im Bau befindlichen Anlagen Advanced Virgo in Italien und Kagra in Japan.
Advanced Ligo wird derzeit weiter optimiert und soll in einem halben Jahr mit voller Leistungsfähigkeit wieder anlaufen. "Ich schätze, dass wir dann alle drei oder vier Tage ein Ereignis wie dieses beobachten werden", hofft Bruce Allen, Geschäftsführender Direktor am MPI für Gravitationsphysik.
Dann werden die Astronomen völlig neue Einblicke in die energiereichsten Vorgänge im Kosmos erlangen. Geo600 kann wegen seiner geringen Abmessungen nur sehr starke Ereignisse messen.
Nach der Entdeckung des Higgs-Teilchens ist dies der zweite fundamentale physikalische Durchbruch in diesem Jahrzehnt. Auch er wird mit dem Nobelpreis belohnt werden. Bleibt nur die Frage, wann und für wen? Für Karsten Danzmann, der seit 1989 an dem Projekt arbeitet, geht die Forschung weiter: "Als Wissenschaftler ist man immer unterwegs und kommt nie irgendwo endgültig an", sagt er und ergänzt: "Jetzt feiern wir aber erst einmal."
Quelle: Die Welt
-
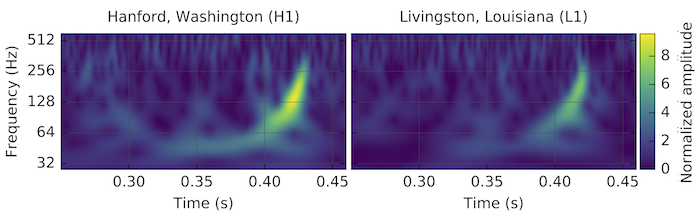
OBSERVATION OF GRAVITATIONAL WAVES FROM A BINARY BLACK HOLE MERGER
Albert Einstein's general theory of relativity, first published a century ago, was described by physicist Max Born as 'the greatest feat of human thinking about nature'. We report on two major scientific breakthroughs involving key predictions of Einstein's theory: the first direct detection of gravitational waves and the first observation of the collision and merger of a pair of black holes.
This cataclysmic event, producing the gravitational-wave signal GW150914, took place in a distant galaxy more than one billion light years from the Earth. It was observed on September 14, 2015 by the two detectors of the Laser Interferometer Gravitational-wave Observatory (LIGO), arguably the most sensitive scientific instruments ever constructed. LIGO estimated that the peak gravitational-wave power radiated during the final moments of the black hole merger was more than ten times greater than the combined light power from all the stars and galaxies in the observable Universe. This remarkable discovery marks the beginning of an exciting new era of astronomy as we open an entirely new, gravitational-wave, window on the Universe.
INTRODUCTION AND BACKGROUND
Gravitational waves are "ripples" in space-time produced by some of the most violent events in the cosmos, such as the collisions and mergers of massive compact stars. Their existence was predicted by Einstein in 1916, when he showed that accelerating massive objects would shake space-time so much that waves of distorted space would radiate from the source. These ripples travel at the speed of light through the Universe, carrying with them information about their cataclysmic origins, as well as invaluable clues to the nature of gravity itself.
Over the past few decades astronomers have amassed strong supporting evidence that gravitational waves exist, chiefly by studying their effect on the motions of tightly orbiting pairs of stars in our Galaxy. The results of these indirect studies agree extremely well with Einstein's theory - with their orbits shrinking, exactly as predicted, due to the emission of gravitational wave energy. Nevertheless the direct detection of gravitational waves as they reach the Earth has been hugely anticipated by the scientific community as this breakthrough would provide new and more stringent ways to test general relativity under the most extreme conditions and open up an entirely novel way to explore the Universe.
In the same year that Einstein predicted gravitational waves, the physicist Karl Schwarzschild showed that Einstein's work permitted the existence of black holes: bizarre objects which are so dense and so compact that not even light can escape their gravitational field. Although by definition we cannot directly "see" light from a black hole, astronomers have gathered a great deal of circumstantial evidence for their existence by studying the effects of black hole candidates on their immediate surroundings. For example, it is thought that most galaxies in the Universe, including the Milky Way, contain a supermassive black hole at their center - with masses millions or even billions of times that of the Sun. There is also evidence of many black hole candidates with much lower masses (ranging from a few, to a few dozen, times the Sun's mass), believed to be the remnants of dead stars that have undergone a cataclysmic explosion known as a core-collapse supernova.
Alongside this substantial progress in the indirect observation of black holes, there have been dramatic improvements in our theoretical understanding of these bizarre objects - including, over the past decade, some remarkable advances in modeling a pair of black holes (referred to as a binary) through several close orbits before they finally merge. These computer models have allowed us to construct precise gravitational waveforms - i.e. the pattern of gravitational waves emitted by the black holes as they approach ever closer and finally merge into a single, larger black hole - in accordance with the predictions of general relativity. The direct observation of a binary black hole merger would therefore provide a powerful cosmic laboratory for testing Einstein's theory.
THE LIGO DETECTORS
LIGO is the world's largest gravitational wave observatory and one of the world's most sophisticated physics experiments. Comprised of two giant laser interferometers located thousands of kilometers apart, one in Livingston, Louisiana and the other in Hanford, Washington, LIGO uses the physical properties of light and of space itself to detect gravitational waves - a concept first proposed in the early 1960's and the 1970's. A set of initial interferometers was completed by the early 2000s, including TAMA300 in Japan, GEO600 in Germany, LIGO in the United States and Virgo in Italy. Combinations of these detectors made joint observations between 2002 and 2011, but did not detect any gravitational wave sources. After undergoing major upgrades, in 2015 the LIGO detectors began operation as Advanced LIGO: the first of a significantly more sensitive global network of advanced detectors.
An interferometer like LIGO consists of two "arms" (each one 4km long) at right angles to each other, along which a laser beam is shone and reflected by mirrors (suspended as test masses) at each end. When a gravitational wave passes by, the stretching and squashing of space causes the arms of the interferometer alternately to lengthen and shrink, one getting longer while the other gets shorter and then vice-versa. As the interferometers' arms change lengths, the laser beams take a different time to travel through the arms - which means that the two beams are no longer "in step" (or in phase) and what we call an interference pattern is produced. This is why we refer to the LIGO detectors as "interferometers".
The difference between the two arm lengths is proportional to the strength of the passing gravitational wave, referred to as the gravitational-wave strain, and this number is mind-bogglingly small. For a gravitational wave typical of what we can detect, we expect the strain to be about 1/10,000th the width of a proton! However LIGO's interferometers are so sensitive that they can measure even such tiny amounts.
Figure 2 shows a simplified diagram of an Advanced LIGO detector.
To successfully detect a gravitational wave event like GW150914 the LIGO detectors need to combine astounding sensitivity with an ability to isolate real signals from sources of instrument noise: tiny disturbances, due to e.g. environmental effects or the behavior of the instruments themselves, that could mimic - or indeed simply overwhelm - the signature strain pattern we are looking for. This is a key reason why there are two Advanced LIGO detectors, as it allows us to distinguish gravitational waves from local instrumental or environmental effects: only a real gravitational wave signal would appear in both detectors - albeit separated by a few thousandths of a second, to account for the time taken for light (or a gravitational wave) to travel between the two detector sites.
Inset (b) in Figure 2 shows how the instrument noise in the LIGO detectors depends on frequency. We can see that the instrument noise is lowest in the "sweet spot" around a few hundred hertz, but increases sharply at both low and high frequencies. There are also a number of narrow spikes where the instrument noise is particularly large, due to e.g. vibration of the fibers that suspend the mirrors and test masses in each interferometer.
Reaching the much greater sensitivity of Advanced LIGO required the upgrading of almost every aspect of the Initial LIGO design. These upgrades included:
Significantly increasing the laser power, to reduce the main source of high frequency noise
Redesigning the recyclying cavities to better contain the spatial distribution of the laser light
Using larger, heavier fused silica test masses, to reduce the random motions of the mirrors
Suspending the test masses using fused silica fibers, to reduce their thermal noise
Suspending the test masses with a four-stage pendulum, improving their seismic isolation
Using an active "measure and cancel" strategy for reducing the impact of ground motions
Operating a network of two or more detectors also lets us "triangulate" the direction on the sky from which a gravitational wave arrives, by studying the difference in arrival time at each detector. The more detectors in one's network, the better the sky position of a gravitational wave source can be localised. In 2016 the Advanced Virgo detector, in Italy, will join the global network, and other advanced interferometers are planned for the future. For more details see e.g. this publication.
OUR LIGO OBSERVATIONS AND WHAT THEY MEAN
On September 14, 2015 at 09:50:45 Greenwich Mean Time the LIGO Hanford and Livingston Observatories both detected a signal from GW150914. The signal was identified first by what we call low-latency search methods that are designed to analyse the detector data very promptly, looking for evidence of a gravitational-wavelike pattern but without modeling the precise details of the waveform. These prompt searches reported the candidate event within only three minutes of the signals arriving at the detectors. The gravitational-wave strain data acquired by the LIGO interferometers was then compared with an extensive bank of theoretically predicted waveforms - a process known as matched filtering - with the goal of finding the waveform that best matched the data.
Figure 3 presents key results of these detailed analyses - all of which firmly point to GW150914 being produced by the coalescence of two black holes. The middle part of the figure shows our reconstruction of the gravitational-wave strain, as seen by the Hanford detector. Note, in particular, the impressive agreement between this pattern (shown in grey) and (shown in red) a waveform for two coalescing black holes consistent with our data, computed using general relativity.
Images of the black hole horizons at various stages of this computation are shown at the top of the figure: the inspiral, as the two black holes approach each other; the merger as the black holes join together and the subsequent ringdown, as the single black hole that has newly formed briefly oscillates before settling down.
Comparing the strain data with theoretical predictions allows us to test whether general relativity is able to fully describe the event. It passes this test with flying colors: all of our observations are consistent with the predictions of general relativity.
We can also use the data to estimate the specific physical characteristics of the system that produced GW150914, including the masses of its two black holes before the merger, the mass of the single post-merger black hole, and the distance of the event.
Our results indicate that GW150914 was produced by the merger of two black holes with masses of about 36 times and 29 times the mass of the Sun respectively, and that the post-merger black hole had a mass of about 62 times the Sun's mass. Moreover, we infer that the final black hole is spinning - such rotating black holes were first predicted theoretically in 1963 by mathematician Roy Kerr. Finally, our results indicate that the GW150914 occurred at a distance of more than one billion light years. So the LIGO detectors have observed a truly remarkable event that happened a long time ago in a galaxy far, far away!
If we compare the masses of the pre- and post-merger black holes, we see that the coalescence converted about three times the mass of the Sun (or nearly six million trillion trillion kilograms) into gravitational-wave energy, most of it emitted in a fraction of a second. By contrast the Sun converts a mere two billionths of one trillionth of its mass into electromagnetic radiation every second. In fact, the gravitational-wave power radiated by GW150914 was more than ten times greater than the combined luminosity (i.e. the light power) of every star and galaxy in the observable Universe.
HOW DO WE KNOW GW150914 WAS A BLACK HOLE MERGER?
Our estimated pre-merger masses of the two components in GW150914 make a very strong argument that they are both black holes - particularly when we also consider the enormous velocity and tiny separation of the two components, as shown in the lower part of figure 3. In this figure indicative velocities of the two components are seen to be significant fractions of the speed of light. Similarly their approximate separation is shown to be just a few times the characteristic size of a black hole, known as its Schwarzschild radius.
These graphs imply that the two components were only a few hundred kilometers apart just before they merged, ie. when the gravitational-wave frequency was about 150 Hz. Black holes are the only known objects compact enough to get this close together without merging. Based on our estimated total mass for the two components, a pair of neutron stars would not be massive enough, and a black hole-neutron star pair would have already merged at a lower frequency than 150 Hz.
ARE WE SURE THAT GW150914 WAS A REAL ASTROPHYSICAL EVENT?
The short answer is "yes", but of course this is a crucial question and the LIGO Scientific Collaboration and Virgo Collaboration have together made a huge effort to address it, carrying out a variety of independent and thorough checks - all of which contribute to strengthening the detection case for GW150914.
Firstly, as we noted already, the time delay between the observations made at each LIGO detector was consistent with the light travel time between the two sites. Also, as seen in figure 1, the Hanford and Livingston signals showed a similar pattern, as would be expected given the near alignment of the two interferometers, and were strong enough to "stand out" against the background noise around the time of the event - like a burst of laughter heard above the background chatter of a crowded room.
Understanding this background noise is an essential part of our analysis and involves monitoring a vast array of environmental data recorded at both sites: ground motions, temperature variations and power grid fluctuations to name just a few. In parallel, many data channels monitor in real time the status of the interferometers - checking, for example, that the various laser beams are properly centred. If any of these environmental or instrumental channels indicated a problem, then the detector data would be discarded. However, despite exhaustive studies, no such data quality problems were found at the time of the event.
But perhaps GW150914 was a rare noise fluctuation, which happened to occur simply by chance with similar characteristics at both sites? To reject this possibility we need to work out just how rare such a fluctuation would be: the less often it could occur by chance, the more confidently we can rule out this scenario in favour of the alternative - that GW150914 was indeed a real gravitational wave event.
To carry out this statistical analysis we used 16 days' worth of stable, high quality detector strain data from the month following the event. GW150914 was indeed by far the strongest signal observed in either detector during that period. We then introduced a series of artificial time shifts between the H1 and L1 data, effectively creating a much longer data set in which we could search for apparent signals that were as strong (or stronger) than GW150914. By using only time shifts greater than 10 milliseconds (the light travel time between the detectors) we ensured that these artificial data sets contained no real signals, but only coincidences in noise. We can then see, in the very long artificial data set, how often a coincidence mimicking GW150914 would appear. This analysis gives us the false alarm rate: how often we could expect to measure such a seemingly loud event that was really just a noise fluctuation (i.e. a "false alarm").
Figure 4 (adapted from figure 4 of our publication) shows the result of this statistical analysis, for one of the searches carried out on our detector data. The solid black and purple curves represent the "background": the number of coincidental noise "events" that we estimate would be produced (under slightly different assumptions) for different strengths of signal. The orange boxes represent what we actually saw, without the artificial time shifts. The key message of this figure is how far away the observed event GW150914 is from the background noise. This means that a noise event mimicking GW150914 would be exceedingly rare - indeed we expect an event as strong as GW150914 to appear by chance only once in about 200,000 years of such data! This false alarm rate can be translated into a number of "sigma" (denoted by s), which is commonly used in statistical analysis to measure the significance of a detection claim. This search identifies GW150914 as a real event, with a significance of more than 5 sigma.
CONCLUSIONS AND OUTLOOK
The first direct detection of gravitational waves and the first observation of a binary black hole merger are remarkable achievements, but they represent only the first page of an exciting new chapter in astronomy.
The next decade will see further improvements to the Advanced LIGO detectors and extension of the global detector network to include Advanced Virgo in Italy, KAGRA in Japan, and a possible third LIGO detector in India.
This enhanced global network will significantly improve our ability to locate the positions of gravitational-wave sources on the sky and estimate more accurately their physical properties. The nascent field of gravitational-wave astronomy has a very bright future!
.
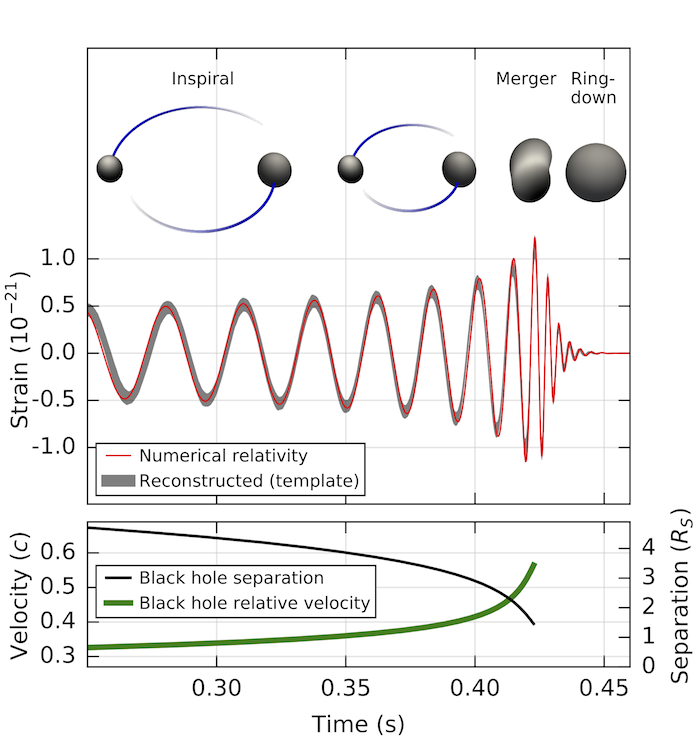
Quelle: LIGO Scientific Collaboration
4503 Views